The plastic crisis has reached staggering proportions. If all the plastic waste on Earth were laid out, it would be enough to cover the entire surface of Argentina ankle-deep.
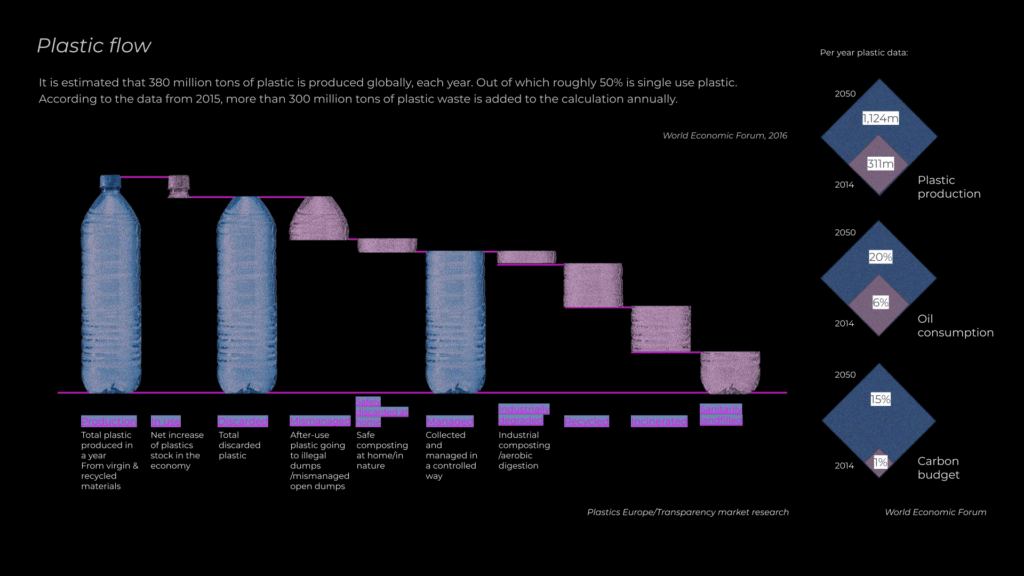
Every year, we produce 300 million tons of plastic globally. Yet only 10% remains in use, while more than 80% is discarded. Of this discarded plastic, just 20% is recycled, leaving the vast majority to accumulate in landfills, pollute the environment, and infiltrate ecosystems as microplastics.
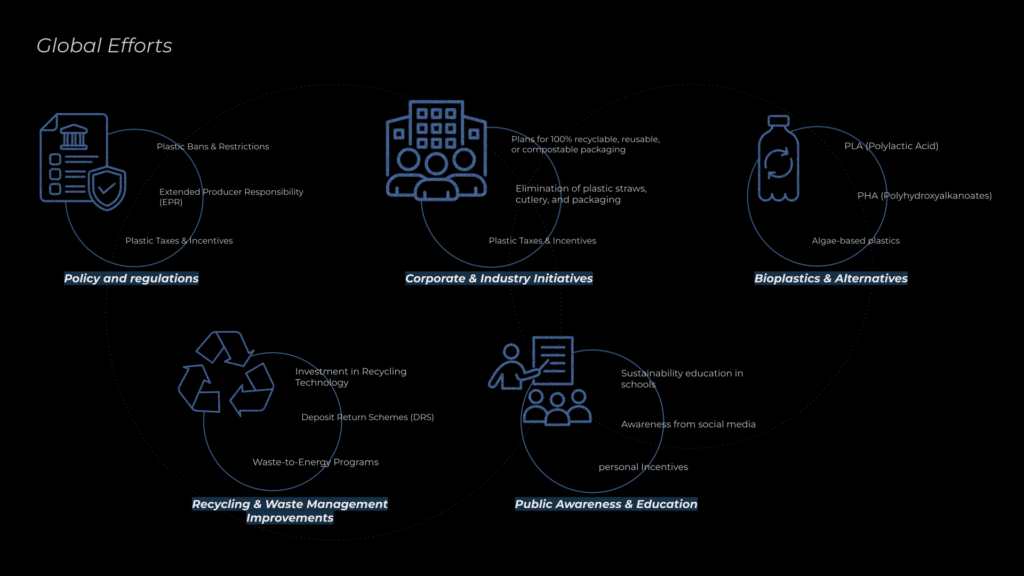
Governments are implementing plastic bans, taxes, and Extended Producer Responsibility (EPR) laws to reduce waste, while industries are adopting biodegradable packaging, refillable systems, and circular economy models. Recycling efforts are improving with advanced sorting, chemical recycling, and deposit-return programs, and public awareness campaigns promote responsible consumption. There has been a rise in fossil fuel plastic alternatives, among them PLA (polylactic acid), is gaining attention as a sustainable replacement in packaging and manufacturing.
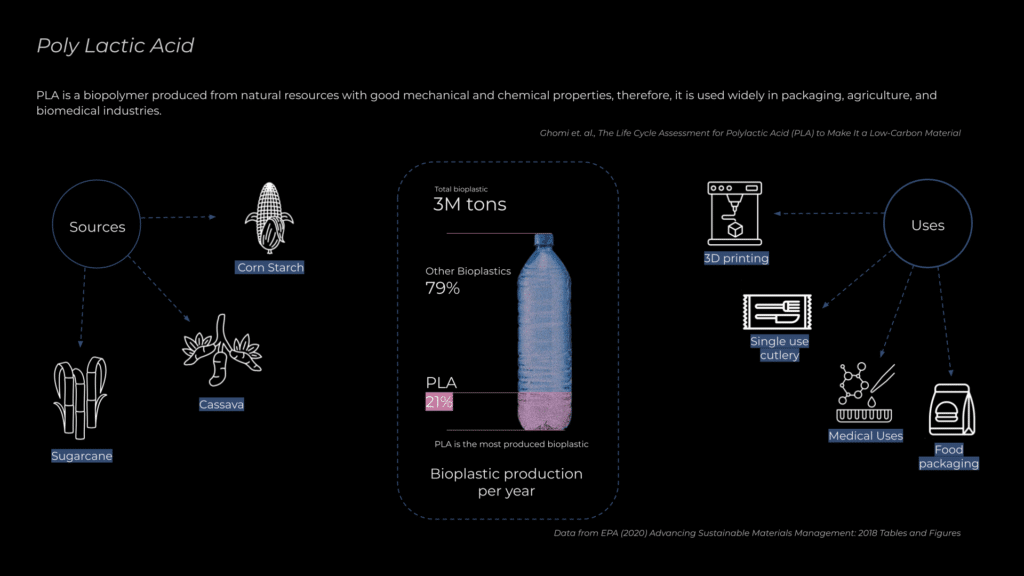
PLA (Polylactic Acid) is a biodegradable and bio-based plastic made from renewable resources like corn starch, sugarcane, or cassava. It is widely used in packaging, disposable cutlery, 3D printing, and medical applications due to its versatility and lower environmental impact since it reduces reliance on fossil fuels.
Polylactic Acid (PLA) is the most widely used bioplastic, accounting for 21% of global bioplastic production. However, while marketed as compostable, PLA only degrades under industrial conditions at temperatures above 60°C. This misleading portrayal contributes to greenwashing, as consumers may wrongly assume it breaks down naturally in any environment.
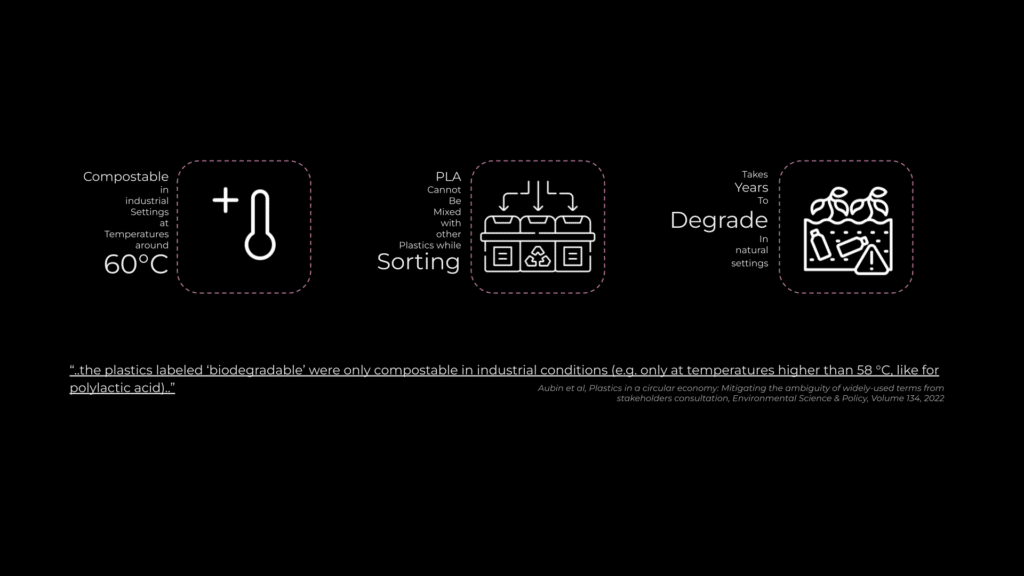
If correctly identified and managed, PLA’s waste management route is disposal, collection, acceptance and treatment at either industrial composting or PLA recycling facilities.
When incorrectly managed, PLA is landfilled, incinerated or littered. In addition, there is a concern that biodegradable or compostable labelling may increase the frequency of littering due to confusion by consumers on what ‘biodegradable’ entails.
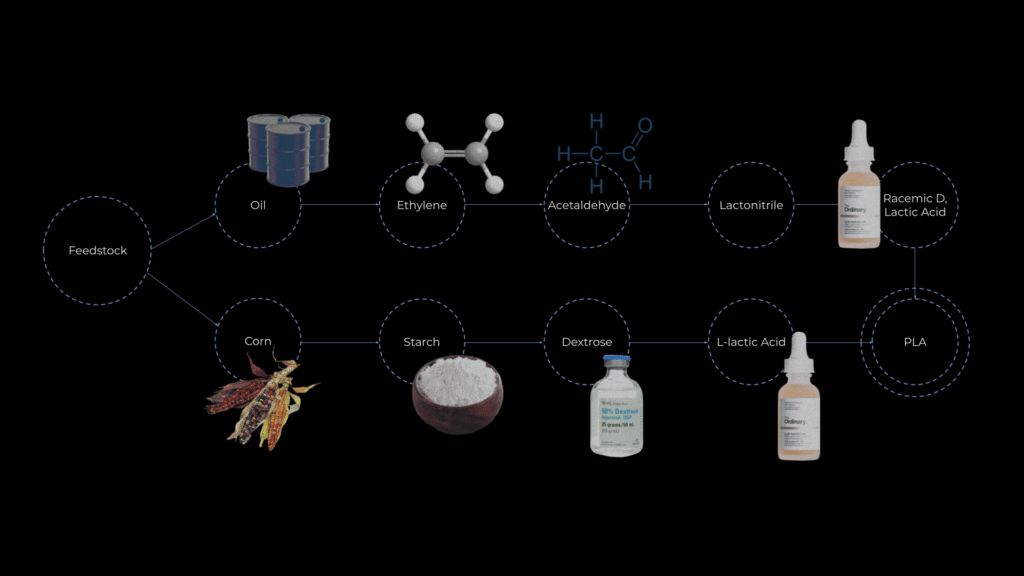
To come up with a solution of how to tackle this problem we need to understand what it actually is, PLA starts at the feedstock level with corn or oil as the starting points and then they get converted into starch or ethylene, then dextrose and actaldyhyde then lactic acid and lactonitrile and then this lactonitrile is converted to lactic acid, lactic acid is also the same chemical that is released in our muscles during anaerobic respiration, when we get tired of running/physical exercise, it is also very popular in skincare products. This lactic acid is then crosslinked and turned into the polymer of polylactic acid PLA.
When PLA degrades it turns back to lactic acid, in industrial composting this lactic acid is further degraded to carbondioxide and water, while we spend energy and resourses to synthesize lactic acid separately. among one of the lactic acid uses is its use as a biostimulant for the soil where lactic acid bacteria, or whey fermentation byproducts are integrated in soil to make lactic acid when then acts as a biostimulant. Though the application of direct lactic acid in soil is unknown. This research aims to find biological means to degrade polylactic acid and find what happens when a shit ton of lactic acid is directly introduced to the soil.
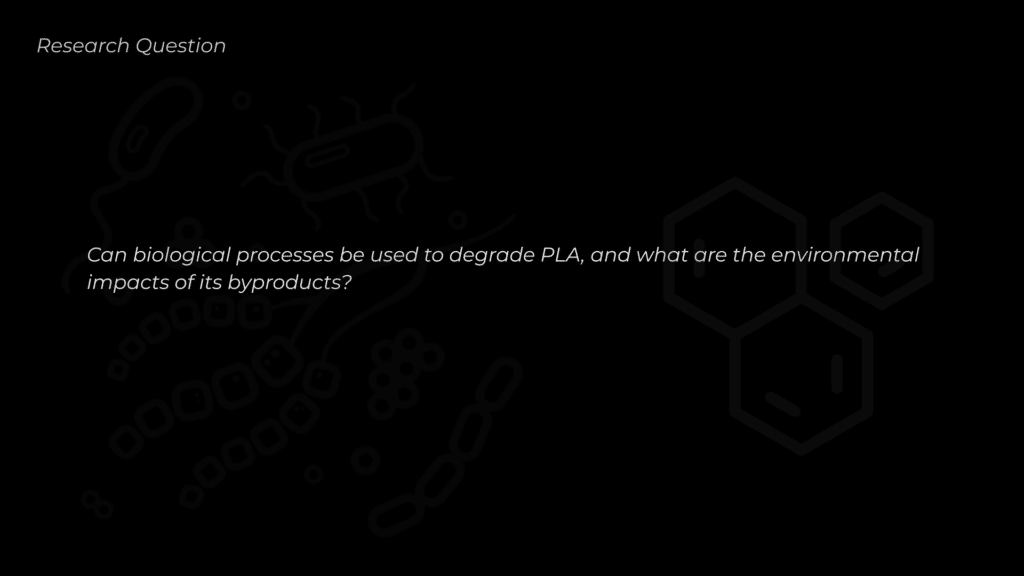
Hence the research question: can biological processes be applied to degrade PLA and what will happen when the byproducts are left in the environment?
Biological processes like Bioremediation, Biofuel Production, etc use plants, algae and microorganisms to our benefit. One of these invlolve fungal and bacterial degradation which offer a sustainable way to break down complex materials without relying on harsh chemicals or industrial processes. Microorganisms can naturally degrade organic compounds, turning waste into harmless byproducts like carbon dioxide, water, and biomass. These processes are self-sustaining, energy-efficient, and adaptable to different environments, making them a viable solution for large-scale waste management. Additionally, biodegrading organisms can be genetically engineered or optimized to target specific materials, speeding up decomposition. By using nature’s own recycling systems, biological degradation could help reduce waste accumulation and promote a circular economy.
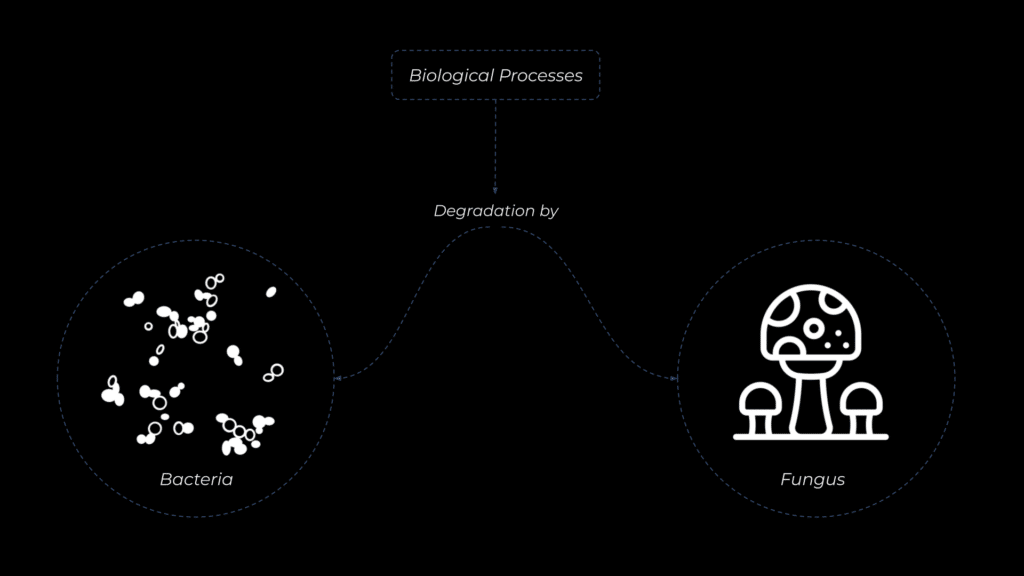
The biological processes I am exploring for PLA degradation involve fungal and bacterial decomposition. While microorganisms naturally break down PLA, the process can take hundreds of years. However, certain microbes can degrade PLA much faster. The challenge is that these microorganisms may not be widespread, abundant, or naturally co-existing in sufficient concentrations to enable efficient degradation.
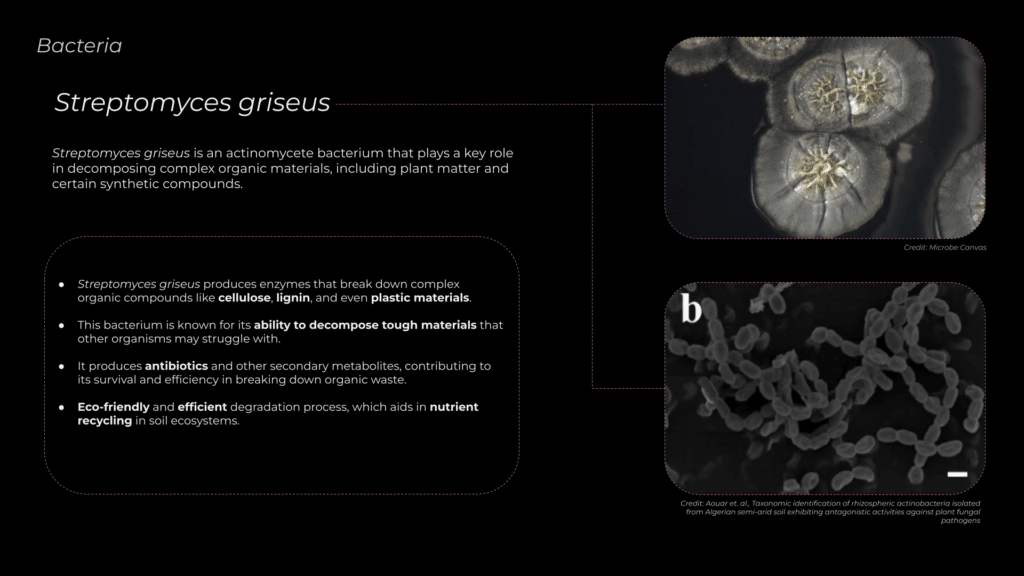
Actinomycetes such as streptomyces grieus Streptomyces griseus is a filamentous bacterium known for its powerful ability to decompose complex organic materials, such as plant fibers (cellulose) and lignin, as well as certain synthetic compounds. This bacterium produces specialized enzymes like cellulase and lignase, which break down tough materials, facilitating their conversion into simpler, harmless byproducts like carbon dioxide, water, and nutrients. Apart from its role in natural decomposition, S. griseus also contributes to nutrient cycling in ecosystems and improving soil health.
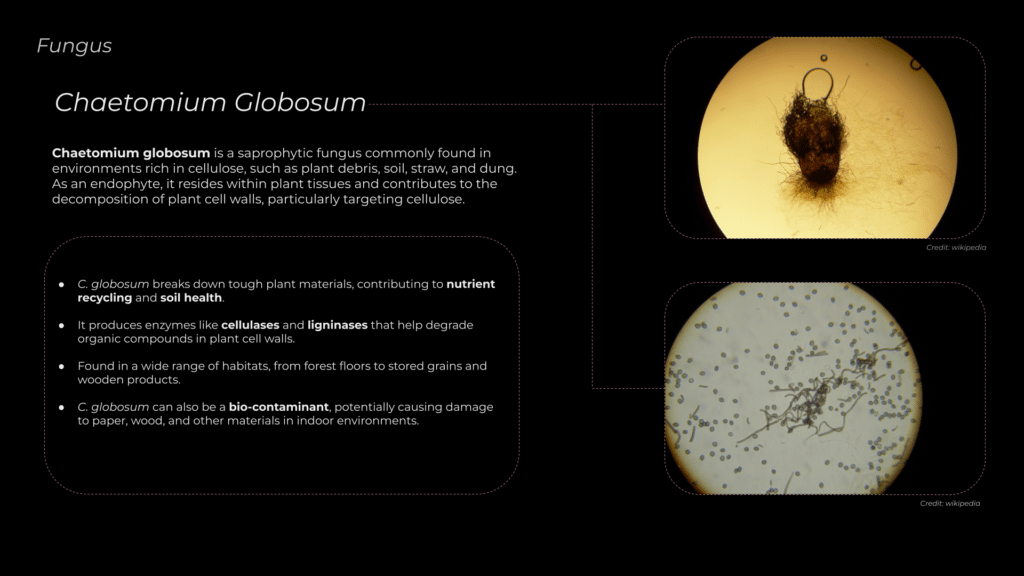
Chaetomium globosum is a saprophytic fungus commonly found in environments rich in cellulose, such as plant debris, soil, straw, and dung. As an endophyte, it resides within plant tissues and contributes to the decomposition of plant cell walls, particularly targeting cellulose.
In natural habitats, C. globosum plays a significant role in breaking down plant material, aiding in nutrient cycling within ecosystems. However, in indoor settings, it can colonize materials like paper and wooden products, leading to deterioration.
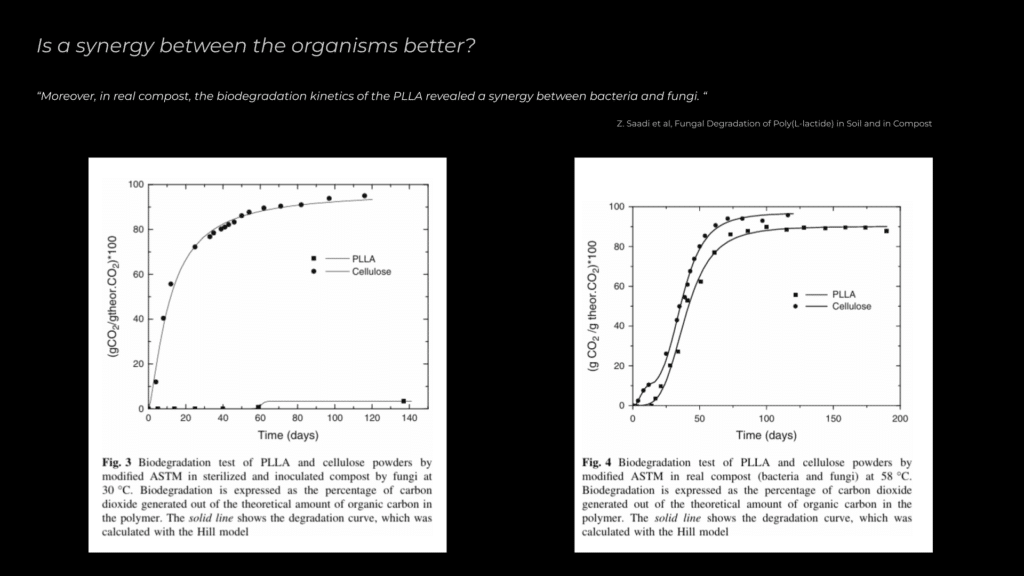
A key aspect of this research is determining whether bacteria, fungi, or a combination of both is more effective in PLA degradation. Saadi et al. found that in real compost settings, the synergy between bacteria and fungi led to the most significant degradation of Poly L-lactic Acid (PLLA). While this finding doesn’t directly apply to my study, it serves as inspiration to explore how a similar interaction might enhance the breakdown of PLA with the microorganisms being investigated.
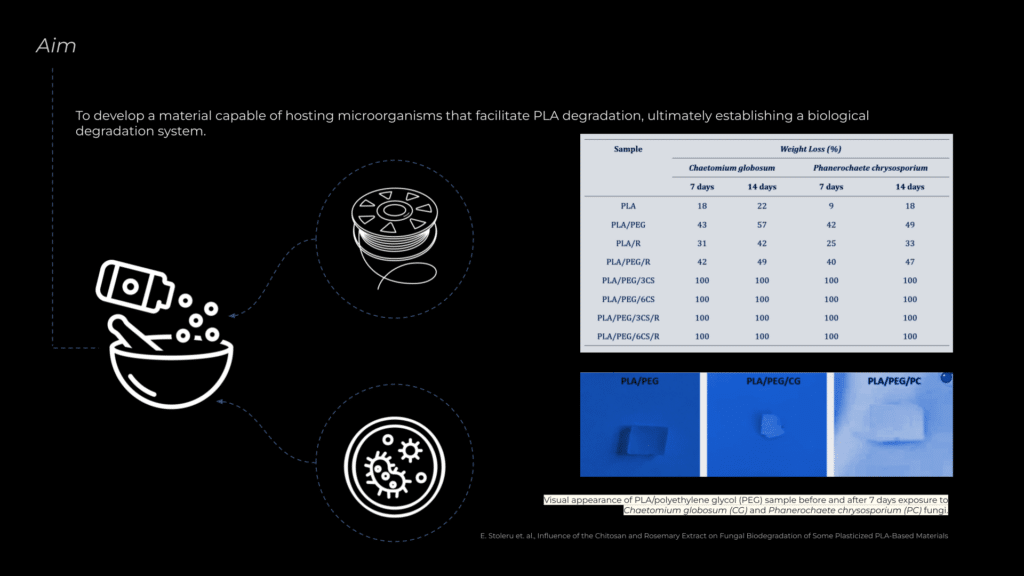
With this understanding, the aim of the research is to develop a material that can host microorganisms alongside PLA, creating a self-sustaining system where PLA is actively degraded and consumed. This process would lead to the complete disappearance of the material, therefore designing an architecture that is transient.
To develop an effective material mix, visiting faculty member and microbiologist Nuria and I reviewed several research papers. One study by Elena Stoleru et al. focused on PLA degradation using microorganisms. They tested various material combinations, and based on their findings, we selected the most efficient mix, incorporating rosemary extract, PEG (polyethylene glycol), and chitosan to enhance microbial activity and PLA breakdown.
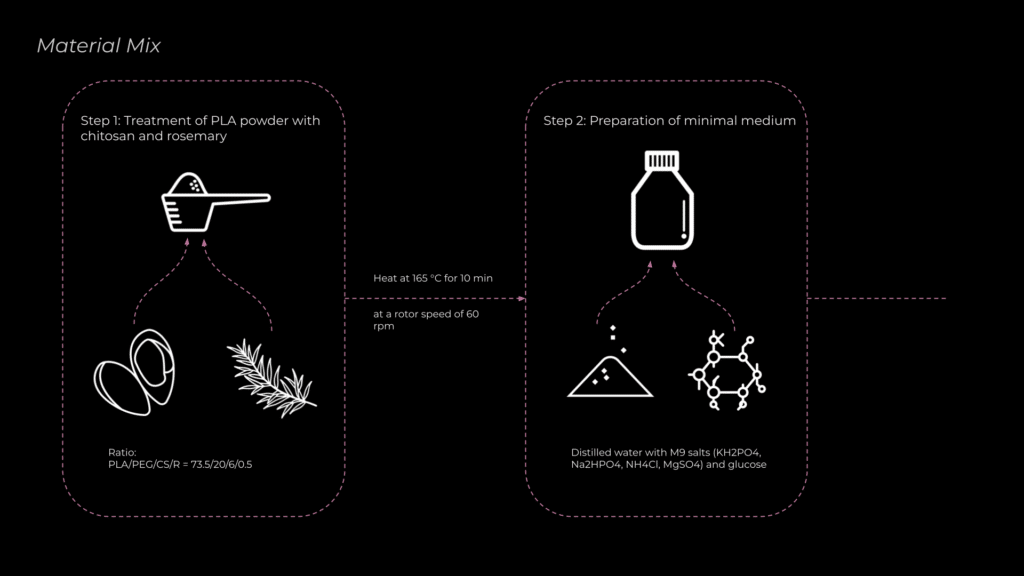
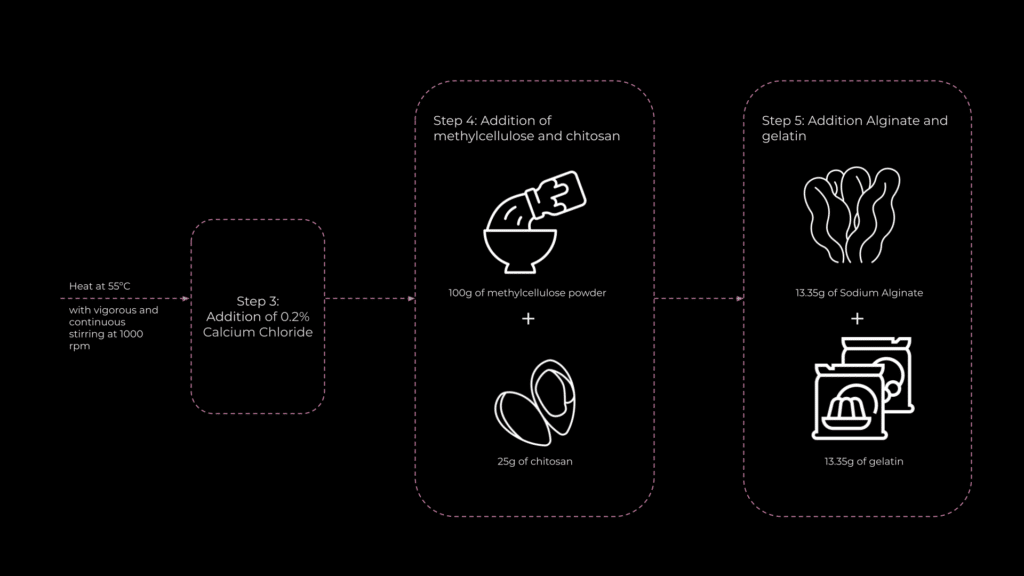
The original method involved heating PLA with rosemary and chitosan, but due to the unavailability of machines capable of controlling mixing speed and temperature, I opted to use unpretreated PLA instead.
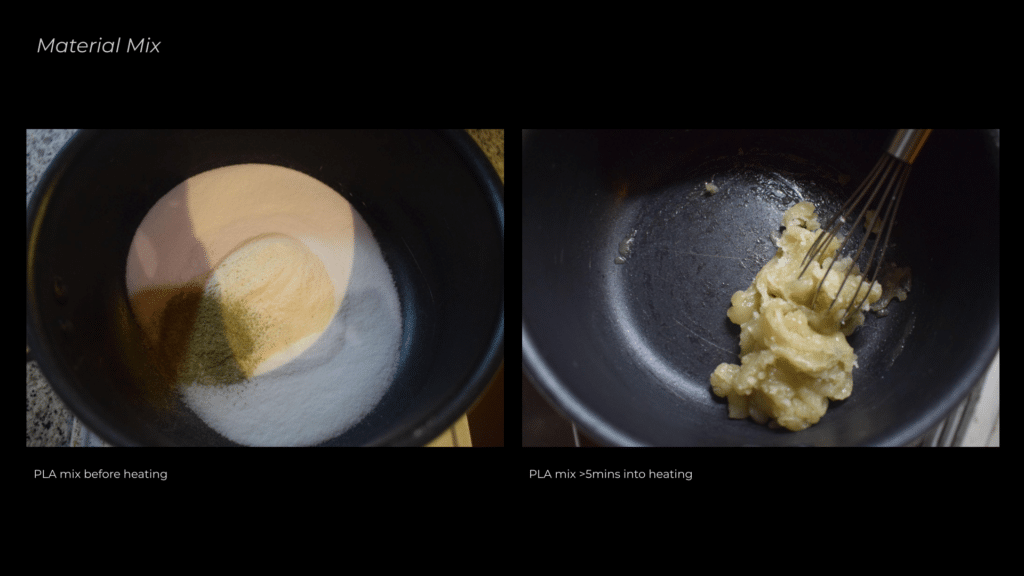
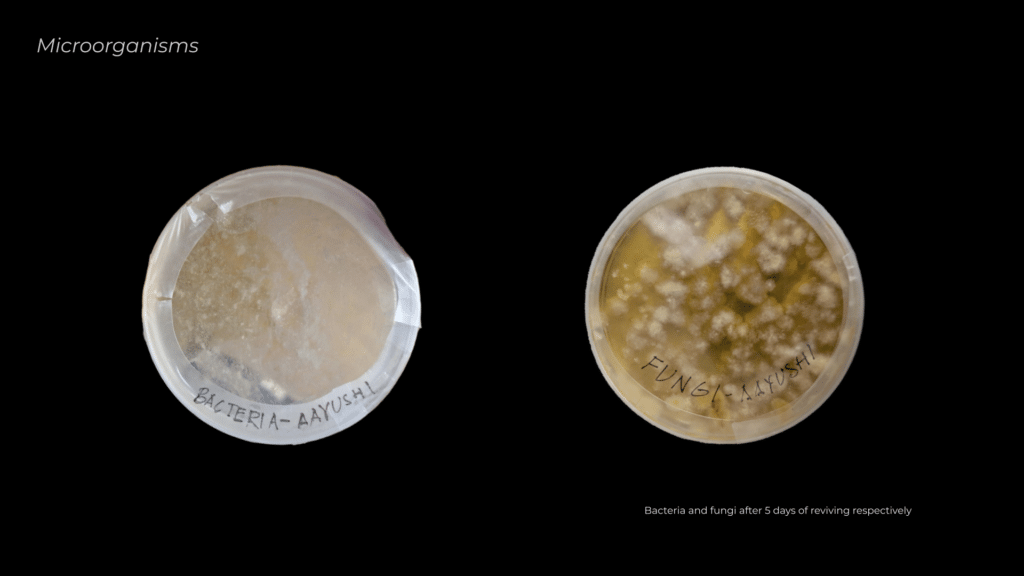
The first step in creating the material mix was to acquire and revive the microorganisms, ensuring they were active and capable of contributing to the PLA degradation process.
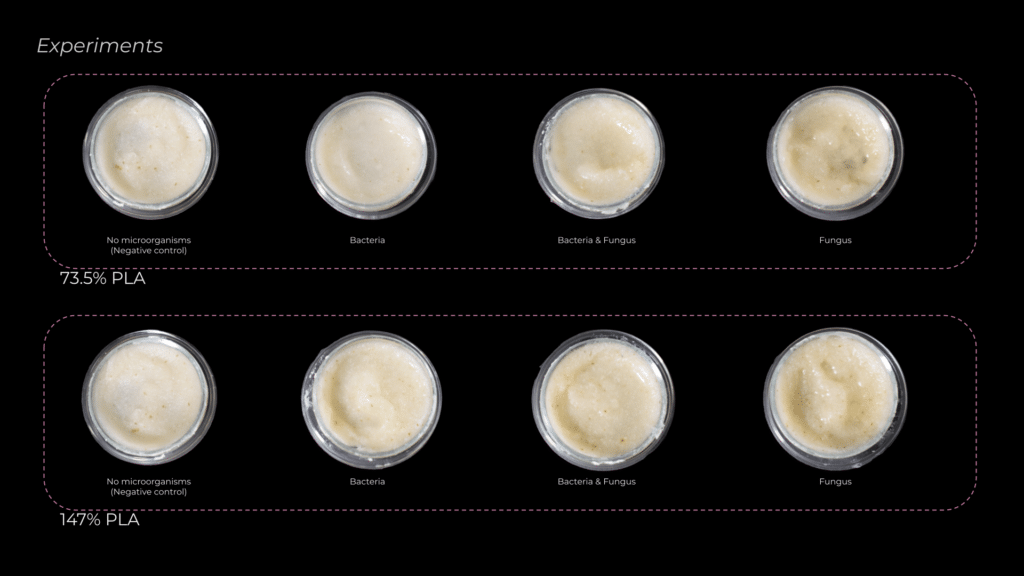
The first experiments I set up aimed to determine whether bacteria, fungi, or a combination of both was more effective in degrading PLA. I also tested different PLA concentrations to observe how degradation efficiency changed. Each PLA percentage had a control petri dish without microorganisms to compare the results.
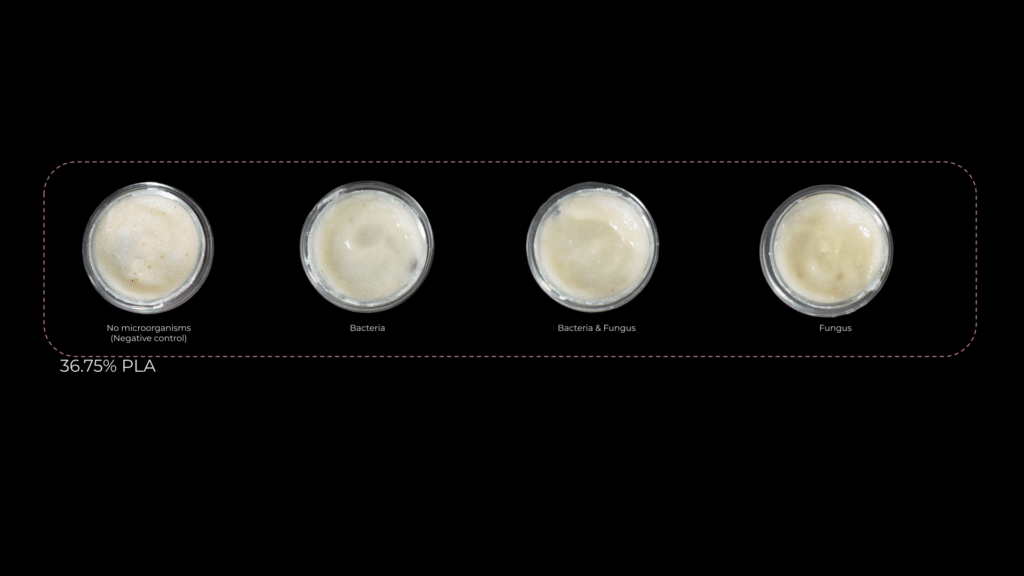
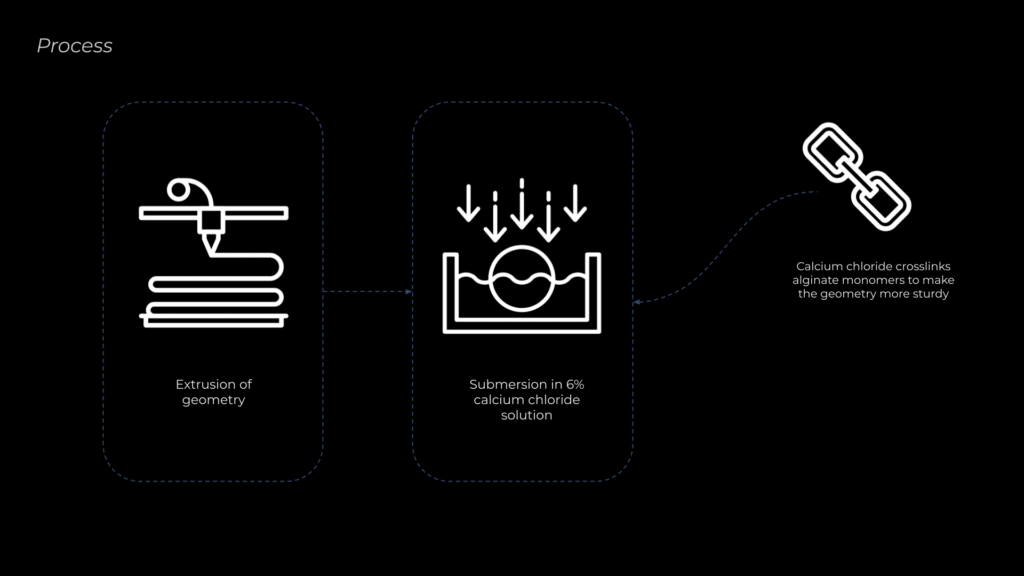
The next step was to refine the fabrication process. To improve stability and crosslink the alginate molecules, the material was submerged in a 6% calcium chloride solution. Initial hand extrusions revealed the need for an extruder capable of gently pushing gel-like materials. For this, I used a syringe extruder developed by former student Ruining Xie.
The first tests involved extruding the material and then submerging it in the calcium chloride solution. However, due to the material’s liquid consistency, the extrusions flattened under gravity. To address this, I experimented with extruding directly into the liquid, introducing the solution after two layers of extrusion and again after five layers, to see if this would help maintain the shape and stability of the material.
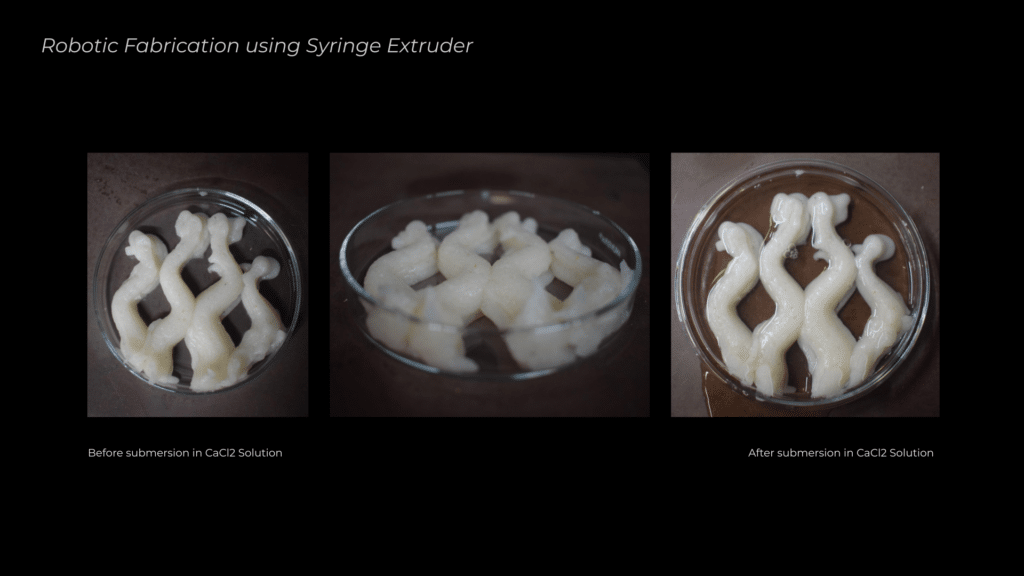
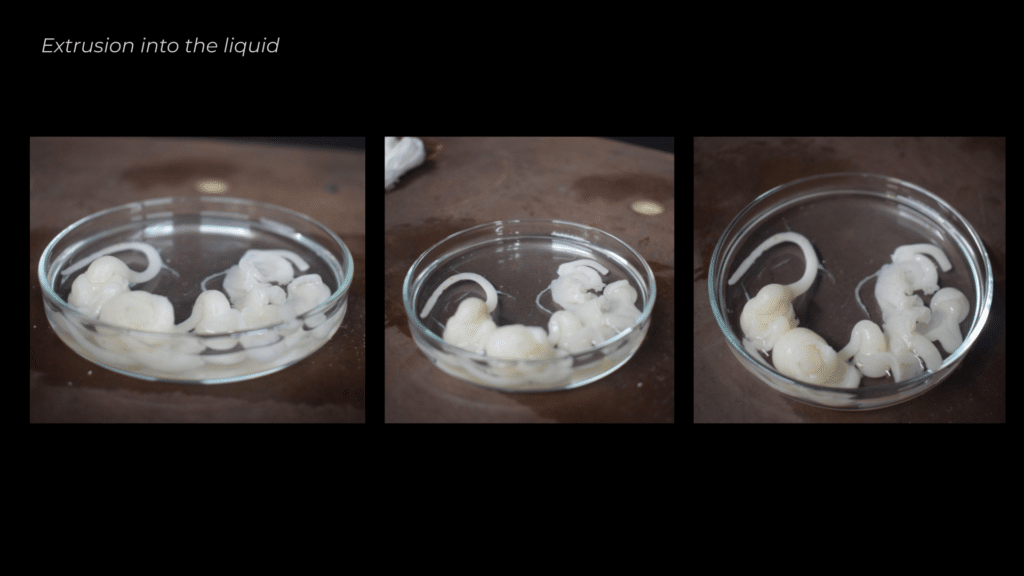
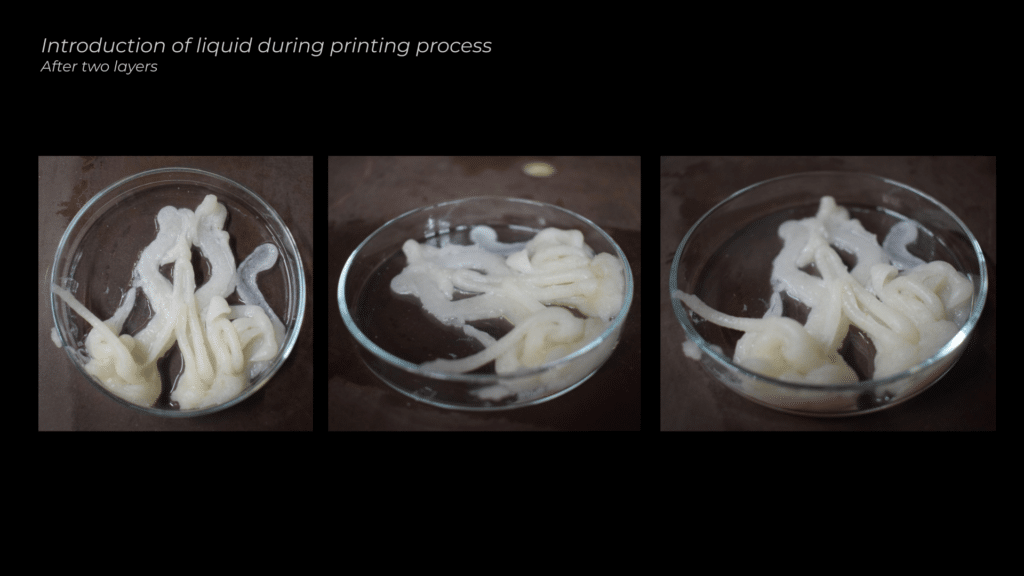
Therefore, I decided to introduce the liquid after two layers of extrusion. However, this approach didn’t work either, as the material didn’t have enough time to stick and dry on the surface before the liquid was added.
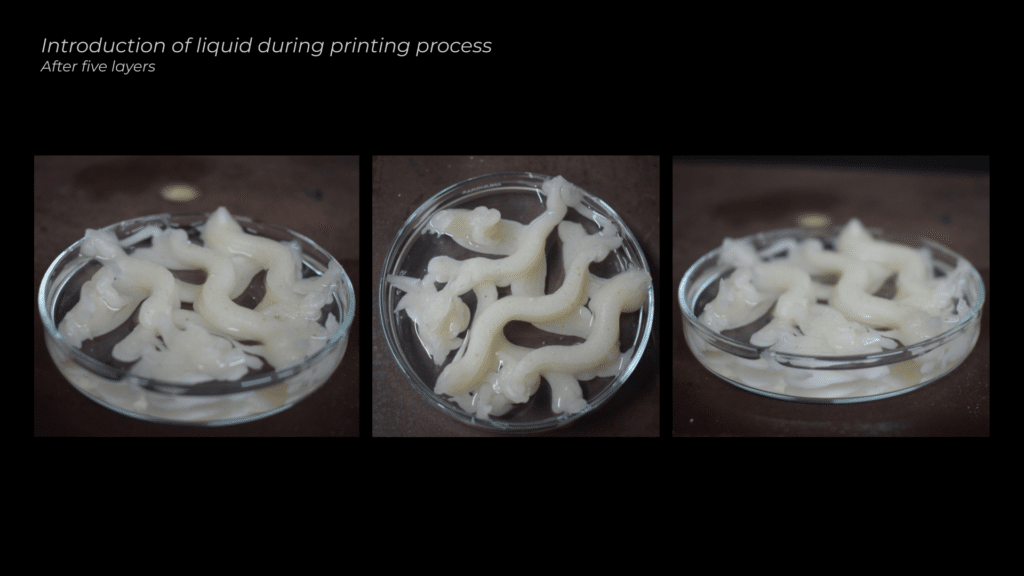
I then decided to wait longer and added the liquid after five layers of extrusion. This method worked halfway, as the lower layers began to adhere, but the upper layers still failed to stick properly to the rest of the model.
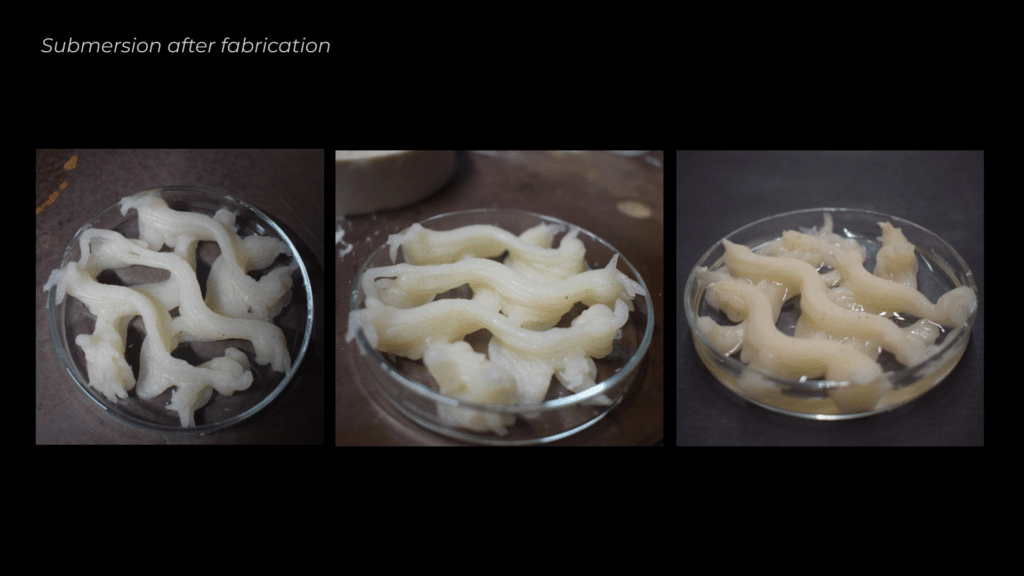
Therefore, I concluded that, although the model might flatten slightly, it’s best to introduce the liquid after the printing process.
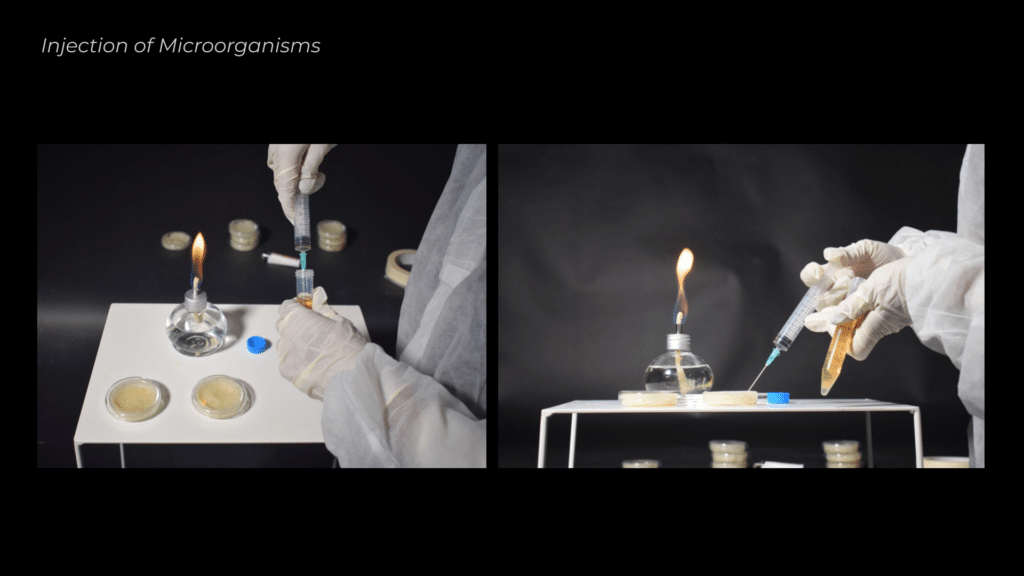
The final step is the injection of microorganisms into the 3D-printed model. The optimal time to introduce the microorganisms is 12 hours after the extrusions are made and the model is submerged. At this point, the material is dry on the outside but still moist on the inside, creating a perfect environment where the microorganisms can be held in a liquid medium, much like being encased in a “skin.”
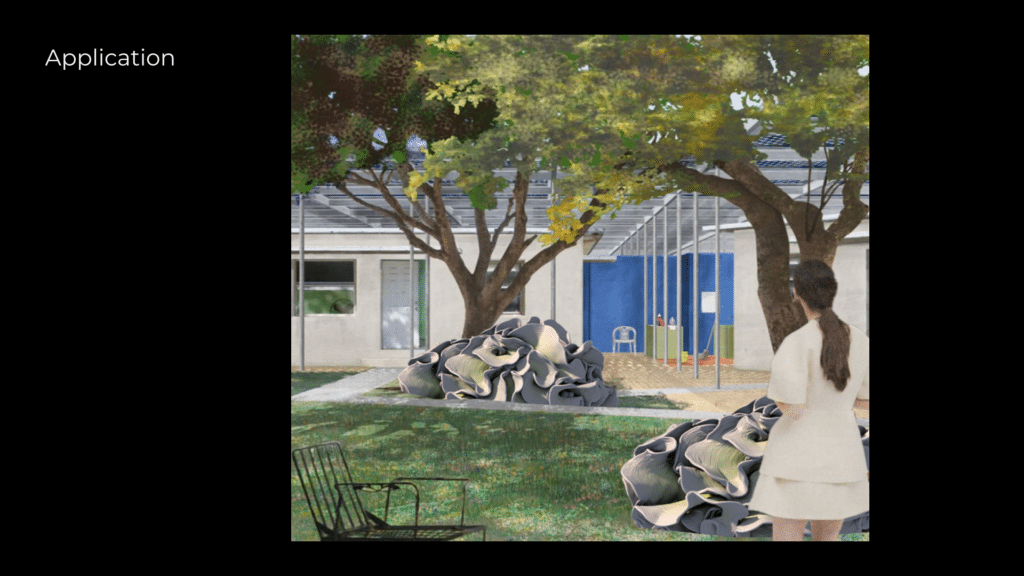
I’m exploring the idea of using this material to create landscape elements and plant pots for areas in need of soil remediation, with the hope that the lactic acid studies will confirm its positive impact on soil health. The next step is to design a geometry that works best for this system. The image above is a conceptual render of this design. Since the fungus requires a geometry that allows for air circulation and isn’t too rigid or straight, this design aims to facilitate the formation of proper hyphal networks, which are essential for effective decomposition.